Investigating the collective behavior of atoms or molecules leading to phases of matter is crucial to understand phenomena such as magnetization, superfluidity, and superconductivity. The laws of quantum physics govern the underlying dynamic of such collective behavior; however, simulating the quantum dynamics involving large number of atoms is often impossible for classical computers.
In 1982, Nobel Prize winner Richard Feynman anticipated that this difficulty might be overcome by using a controllable quantum system to study another less accessible quantum system. This controllable quantum system we can use to understand other physical situations is known today as a quantum simulator.
Scientists from Université Paris-Saclay and The French National Centre for Scientific Research (CNRS), in collaboration with other institutions [1], including scientists from PASQAL, investigated phases of matter in magnetic materials at the atomic level using a neutral atoms quantum processor. They successfully used rubidium atoms (ranging from 42 to 100 qubits) to emulate the quantum dynamics in materials leading to two different magnetic phenomena known as ferromagnetism and anti-ferromagnetism.
Ferromagnetism is present in iron, cobalt, nickel, some rare-earth elements, and specific alloys. Examples of anti-ferromagnetic substances are manganese oxide, chromium, and nickel oxide.
These new experimental results for the 42 qubits were fully comparable with a simulation for the same system performed by the Research Computing Group at Harvard University and at the University of California, Berkeley.
“Successfully benchmarking that kind of complex experiment can require very detailed simulations. We usually start at a small system size, with four qubits arranged in a square, and make sure we understand everything happening during the quantum dynamics, then we scale up the simulations to 42 qubits. At this point, we needed to make some approximations to be able to run them in reasonable times in a computer cluster,” clarifies Lucas Leclerc, Quantum Software Engineer at PASQAL, who was directly involved in the experiment.
However, none of the state-of-the-art classical computers involved in this study could simulate the dynamics for the 100 atoms case. Although it was impossible to compare with classical simulations, the experimental observations using 100 qubits are consistent with theoretical expectations. These results were published in Nature.
But what are ferromagnetic or anti-ferromagnetic materials? And how can we use rubidium atoms to recreate the quantum collective behavior of iron or chromium atoms and the emergence of these magnetic phases in matter? Let’s dive into the details.
Atoms as tiny magnets
Ferromagnetic materials can be turned into magnets by applying an external magnetic field, and their magnetic properties remain even after the external magnetic field is turned off. This behavior comes from their fundamental properties at the atomic level. In these materials, their atoms act as tiny magnets — a quantum property called spin — due to their internal electronic structure. When the external magnetic field is applied, it influences the spins and since they are close enough to each other in the material, each spin also influences their neighbors reinforcing each other, getting aligned all together, turning the material into a magnet itself.
To illustrate ferromagnetism, imagine that we represent the atomic spin with arrows (like the magnetized arrow in a compass). In the ferromagnetic phase, all the heads of the arrows can be found pointing in the same direction with the same probability.
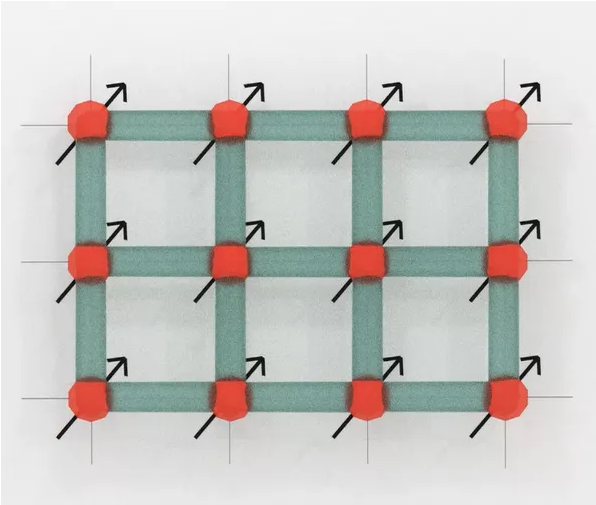
This kind of magnetism is destroyed at high temperatures. When the material is heated to a certain temperature called the Curie point, different for each material, it ceases to be a magnet.
In anti-ferromagnetism, the atoms also act as tiny magnets but, in this case, at low temperatures, they spontaneously align in opposite directions so that their magnetism cancels out and the material does not exhibit any macroscopic magnetic properties. This antiparallel collective behavior disappears upon heating the material to a specific temperature called the Néel point.
Again, imagine the spins as arrows; their heads would alternate in opposite directions in the anti-ferromagnetic phase.
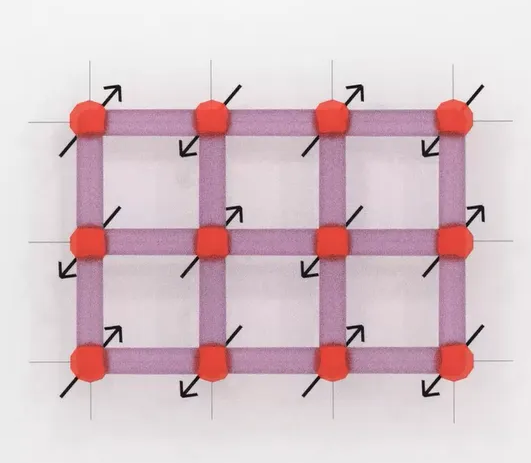
Emulating the emergence of ferromagnetism and anti-ferromagnetism with qubits
In their work, Antoine Browaeys, Thierry Lahaye, and colleagues used neutral rubidium atoms, each of them trapped with intense fine-tuned lasers called optical tweezers, to recreate a quantum model — known as the XY model — of how two-dimensional ferromagnetism and anti-ferromagnetism emerge in nature.
With these optical tweezers, the scientists placed the rubidium atoms in a square array — one with 42 qubits and the other with 100 qubits — representing how atoms in the ferromagnetic material would be organized. Then, they encoded the ferromagnetic material atomic spin by bringing the rubidium atoms to their Rydberg states. Rydberg states are very high-energy electronic states where atoms get polarized, behaving as tiny dipoles. Each of these dipoles interacts with its neighbors, recreating the way the atoms in the original ferromagnetic material interact with their neighbors.
At this point, the dipoles can be oriented in any direction. With this experimental setup, the scientists emulated the quantum dynamics happening in a magnetic material, in two dimensions, before ferromagnetism or anti-ferromagnetism emerged.
By shining light of specific frequencies onto each of the dipoles and controlling that there is no heat transfer in the process, they brought the array to the ferromagnetic phase where all the dipoles get aligned, with the heads of the arrows pointing in the same direction all at once. This model is fully quantum, and because we can only calculate probabilities in quantum physics, these spins, pointing all in the same direction, can be measured to be in any direction with equal probability.
The scientists repeated the experiment, but this time starting from a different energy state and driving the system to the anti-ferromagnetic phase, with the heads of the neighboring arrows pointing in antiparallel directions, again in any antiparallel direction with the same probability.
High-quality science leading to disruptive technologies
In a previous paper, also published in Nature, Antoine Browaeys, Thierry Lahaye, and collaborators already demonstrated their capacity to realize a simpler model, known as the Ising model, of anti-ferromagnetism using 196 qubits. However, that experiment model did not require the level of control needed for this new experiment, where they realized the full quantum XY model, for which the scientists developed new technologies that significantly improved their control over each qubit with high fidelity.
“For me, as an experimentalist, an important aspect in this experiment, beyond the physics results themselves, is that we were able to put together all these new tools, these new lasers, that allowed us to achieve a high level of control over each atom in the array with high fidelity,” explains Thierry Lahaye, Researcher at the French National Centre for Scientific Research (CNRS), and co-founder of PASQAL.
This experimental advance is a big step forward in improving quantum simulations for systems with many atoms and in developing the quantum computer’s fidelity, where we need absolute control of every single atom.
This novel experiment performed at the Université Paris-Saclay in France opens the door to exploring the quantum dynamics of many-body interactions in a programmable quantum simulator in situations where traditional classical methods fail. Innovations in quantum computing will enable us to understand real-life problems, such as in material science or molecular medicine.
PASQAL’s technology is based on high-quality scientific knowledge produced together with our collaborators from world-class institutions. Last year, we demonstrated our capacity to control hundreds of neutral atoms in our quantum processors, and we are now on a solid road map to achieve thousands of qubits.
References
Chen, C., Bornet, G., Bintz, M. et al. Continuous Symmetry Breaking in a Two-dimensional Rydberg Array. Nature (2023). https://doi.org/10.1038/s41586-023-05859-2. Preprint available: 2207.12930.pdf (arxiv.org).
[1]Full list of institutions: Universite Paris-Saclay, Institut d’Optique Graduate School, CNRS, Laboratoire Charles Fabry, University of California, Berkeley, PASQAL SAS, California Institute of Technology, Nanomaterials and Nanotechnology Research Center (CINN-CSIC), Universidad de Oviedo (UO), Technical University of Munich, Munich Center for Quantum Science and Technology (MCQST), Universitaet Innsbruck, Laboratory for Theoretical and Computational Physics, Paul Scherrer Institute, Institute of Physics, Ecole Polytechnique Federale de Lausanne (EPFL), Lawrence Berkeley National Laboratory, Berkeley, Harvard University.